There are 2 types of cloud chambers : the Wilson expansion cloud chamber (1912) and the Langsdorf diffusion cloud chamber (1939). The Wilson chamber did most of the discovery of particles which originated in cosmic rays, until 1950. The diffusion chamber didn’t make such discovery because it only worked in an horizontal plane. As the cosmic particles come « from above », this chamber was not suitable for it. This one was principally used with accelerators, as the incoming particle are produced in an horizontal plan. But diffusion chamber was later superseded in 1952 with a new type of detector, the bubble chamber. Then with the advent of modern particle detectors coupled with computers able to treat a large volume of data, cloud chambers disappeared.
To understand how the cloud chambers contributed to the particles physics, we have to remember what were the available particles detector in the last century.
Particles detector available between 1900-1950
1896 : Photographic plates
Glass photographic plate invented in 1851 where used to capture energetic radiations such as X-rays or α,β particles.
Discovery of X-ray. On the evening of 8 November 1895, while working in a carefully darkened room, Rontgen noticed that a piece of a cardboard coated with barium platinocyanide showed a faint, flickering, greenish light (fluorescence) when electrical discharges took place in a Hittorf-Crookes tube near the screen. The tube itself was carefully covered with a black shield impervious to any visible light. Rontgen verified that the tube was the source of a new kind of radiation, which was invisible but which revealed its existence when hitting the luminescent screen. Rontgen subsequently performed many careful experiments on the radiation which he named X-rays. His first important step was to replace the fluorescent screen by a photographic plate- this was susceptible to X-rays and thus provided a tool for recording X-ray pictures.
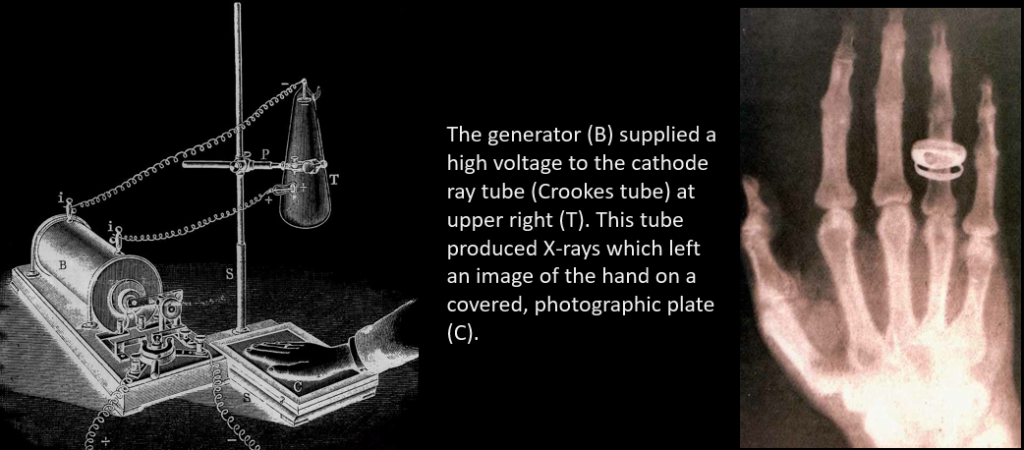
Discovery of radioactivity. February 26, 1896, was an overcast day in Paris — and that presented a problem for French physicist Antoine Henri Becquerel. Becquerel was hoping to demonstrate a link between minerals that glow when exposed to strong light and a new type of electromagnetic radiation called X-rays. The weather thwarted this experiment — but that failure inadvertently produced an entirely new discovery: natural radioactivity.
Becquerel was interested in the phenomenon of fluorescence, in which some materials glow when exposed to sunlight. Physicist Wilhelm Röntgen had recently discovered X-rays; Becquerel thought the two phenomena might be connected, and had designed an experiment of his own. He planned to expose a fluorescing material to the sun, and then place it and a metal object over an unexposed photographic plate. If the developed plate showed the image of the object, he concluded, that would suggest that fluorescing materials are actually emitting X-rays.
But the next day was cloudy as well, and Becquerel was forced to postpone his experiment. He wrapped his fluorescing crystals — a uranium compound called potassium uranyl sulfate — in a black cloth, along with the photographic plate and a copper Maltese cross, and waited for a sunnier day.
Several days later, when Becquerel finally removed the plate from the drawer, he discovered to his surprise that a distinct image of the cross appeared on the plate — although it had never been exposed to sunlight (image below, at left). The only conclusion was that the crystals themselves were emitting radiation. Excited by this prospect, Becquerel decided to repeat the conditions of his unintentional experiment: He again placed a crystal of uranium salt on a photographic plate; he also experimented with putting a crystal on a photographic plate with a sheet of aluminum between, and with a sheet of glass.
After being placed in the dark for several hours, all three plates were blackened by radiation (the crystal in direct contact with the plate showed the strongest blackening). “I am now convinced that uranium salts produce invisible radiation, even when they have been kept in the dark,” he wrote in his diary of his experiments.
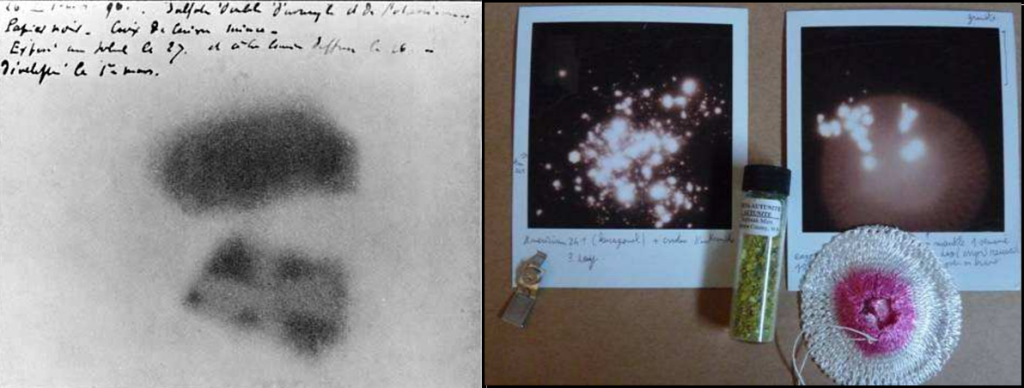
You can make you own photograph of radioactive source using a Polaroid film. The above, right picture show the radioactive print of a Thorium mantle and some autunite mineral, after an exposition of several days in a dark place.
1896 : Electrometer and Ionisation chamber
An electrometer is an electrical instrument for measuring electric charge. The first electrometer come from 1787 (Gold-leaf electroscope). Basically, it’s a voltmeter : the deflection of a needle is proportionnal to a difference of potential applicated to the electrometer. It wasn’t until the development of the Dolezalek electrometer in 1896 that a device was available with the sensitivity needed to measure the very small currents (picoamps) associated with the ionization chambers used to measure radioactive samples. This electrometer used a suspended moving vane (or needle) inside, but not in physical contact with, a metal “pill box” shaped device consisting of four quadrants. Each quadrant was electrically connected to the quadrant diagonally opposite it so that they had the same charge. One pair of quadrants had a positive charge and the other pair had a negative charge. The electrical charge on the vane caused it to take up a particular orientation within the quadrants. If the potential difference between the vane and the quadrants changed, the vane and the mirror would rotate.
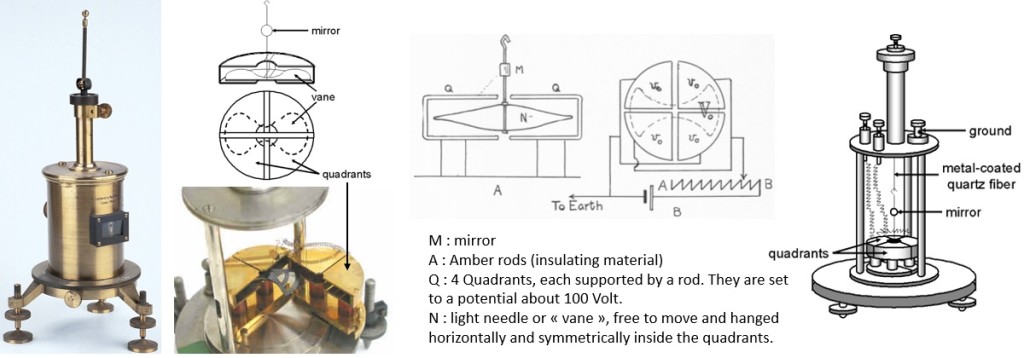
To determine the position of the vane, a beam of light was shone through the window in the electrometer case so that it reflected off the mirror onto a scale usually positioned one meter away. As the current from the ion chamber changed the potential difference between the vane and the quadrants, the vane rotated and the reflected beam of light moved across the scale. The time requited to move across a specified number of divisions on the scale could be related to the activity of the sample by calibration with a known source.
A device was needed to charge the needle of the electrometer : a current source, coming from an ionisation chamber, submitted at the effect of a radioactive source.
Within a couple of months of Roentgen’s discovery of X-rays, J.J Thomson demonstrated that X rays could make a normally insulating material, such as air, conductive. With Rutherford in 1896, they made an experiment in which they exposed a gas to X-Rays. The irradiated gas was blowing to an electrically charged electrode, connected to a quadrant electrometer. They show that the charge of the electrode was leaking and concluded that X-rays can ionize gas (as ions conduct electricity, the charged wire was discharged to the ground by the ionized gas). Plus, the rate of leak of charge from the initially charged central electrode could serve as a measure of the X-rays intensity. At first their ion chamber was employed to measure the intensity of X-rays beams, but Rutherford soon extended this technique to the analysis of uranium and other radioactive materials (1899).
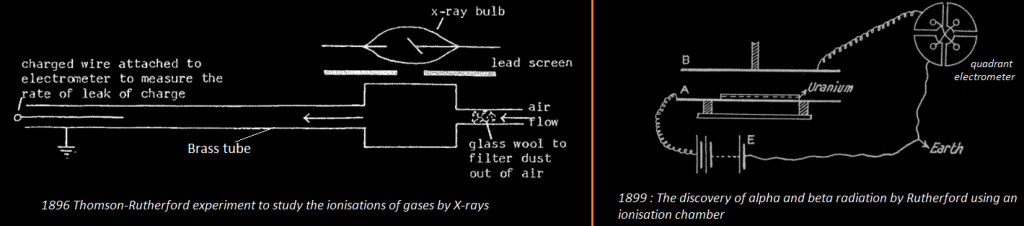
Rutherford used an electrometer to measure an electric current created by the radiation of Uranium in an ionization chamber. A uniform layer of powered uranium compound was spread on plate A and the rays allowed to ionize the gas between plates A and B. The amount of ionization is measured by the « saturation current » received at B when the potential difference between A and B is great enough to pull all the ions to the plates before they are able to recombine.
Rutherford then proceeded to cover the uranium with aluminum sheets of various thicknesses and measure the current, using the electrometer and found that there were at least two different « rays » being emitted by the uranium. He called them α and β.
1903: Spinthariscope
The first instrument that was able to detect individual rays was the spinthariscope, invented by Crookes in 1903. While observing the apparently uniform fluorescence on a zinc sulfide screen created by the radioactive emissions (mostly alpha radiation) of a sample of radium bromide, he spilled some of the sample, and, owing to its extreme rarity and cost, he was eager to find and recover it. Upon inspecting the zinc sulfide screen under a microscope, he noticed separate flashes of light created by individual alpha particle collisions with the screen. Crookes took his discovery a step further and invented a device specifically intended to view these scintillations. It consisted of a small screen coated with zinc sulfide affixed to the end of a tube, with a tiny amount of radium salt suspended a short distance from the screen and a lens on the other end of the tube for viewing the screen. Crookes named his device from Greek « spark ». You can build a spinthariscope using an Am241 source and a ZnS:Ag screen available on ebay.
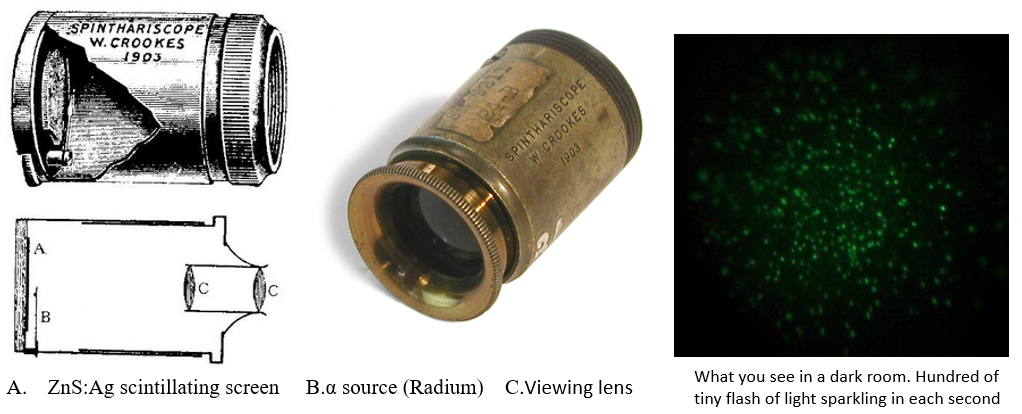
Thus a very simple method for counting individual alpha particles was available (but it imposed considerable strain and fatigue on the observers). The method was refined and used most profitably by Rutherford and his students and was extensively used for many years until the 1930s. However Rutherford said about this visual counting method :
In considering a possible method of counting the number of a-particles, their well-known property of producing scintillations in a preparation of phosphorescent zinc sulphide at once suggests itself. With the aid of a microscope, it is not very difficult to count the number of scintillations appearing per second on a screen of known area when exposed to a source of a-rays. The doubt, however, at once arises whether every a-particle produces a scintillation, for it is difficult to be certain that the zinc sulphide is homogeneous throughout.
So in 1908 Rutherford & Geiger found an alternate method for counting single alpha particle, without the fatigue of observation and possible losses due to the quality of the scintillating screen.
1908: Rutherford-Geiger Counter
Until 1908 the intensity of radiation was measured with an ionisation chamber coupled to an electrometer. This device was insensitive to single rays and can only detect « bulk » radiations. Rutherford wanted to measure the current induced by a single alpha particle (in order to estimate the number of alpha particles produced by a radioactive compound, and thus, calculating it’s mass activity). The detection of a single particle producing a small direct electrical effect in the ionisation chamber, would require a very sensitive electrometer, isolated from any disturbance. As the current is very low, the movement of the electrometer’s needle is very thin (about 0.3 mm) thus the repeatability and the fiability of this counting method is not demonstrated.
Rutherford and Geiger in 1908 produced a remarkable article about a new electrical method of counting. This method automatically magnified several thousand times the electrical effect due to a single α-particle which gave easily measurable movement of the needle of an ordinary electrometer. They still used an ionisation chamber but this time the electric field between the electrode was very high, just below the dielectric breakdown of air and the pressure inside the chamber was few mm of mercury (previous ionization chamber were at atmospheric pressure, 760 mm Hg). This apparatus follow the research of J.S Towsend who found the Avalanche Effect in 1902.
This counter was the first approach of the final version of the Geiger-Muller counter of 1928 as a practical instrument. The apparatus of 1908 was not widely used as a counter because of it’s unexplained spurious discharges in absence of alphas. This was interpreted as an instability of the instrument as it’s was not clear if a discharge came from a ‘real’ counting events of α– or β-radiation or if it’s was due by the instrument itself. In 1928 it was discovered by Muller, who isolated the device from the exterior, that these unexpected discharges were triggered by cosmic rays (discovered in 1912). So in 1928, Geiger-Muller improved the apparatus to a stable experimental setup with reliable and reproducible results.
1911 : Wilson Expansion Cloud Chamber
In 1895 C. T. R. Wilson wanted to reproduce in the Cavendish laboratory the rainbow halo that he saw during a trip on the summit of Ben Nevis (picture below). He made some experiments for this purpose – making clouds by expansion of moist air after the manner of Aitken. He found that after dust particles are removed, cloud formation will not occur until the volume expansion ratio equals 1.25. If the expansion ratio was beyond 1.25, dense clouds were formed in the dust-free air. The number of drops in the shower showed no diminution however often the process of producing the shower and allowing the drops to fall was repeated. It was evident then that the nuclei were always being regenerated in the air. Note : we know nowadays that theses « nuclei » are in fact ions which act as cloud condensation nuclei (like dusts and aerosols). These ions are produced continuously in the atmosphere with the cosmic rays, and are able to discharge electroscope, a mystery of early 1900 which was solved in 1912 with the discovery of cosmic rays.
In early 1896, few months after the discovery of X-rays and using a primitive form of X-rays tube, he exposed X-rays to his cloud chamber : this greatly increased the number of droplets during an expansion. The X-rays produced more « nuclei » of the same kind as were always being produced in very small numbers in the air within the cloud chamber. In 1899, he demonstrated that the condensation nuclei produced by X-rays were shown to be ions by their behavior in an electric field. He also noticed that the least expansion required (1,25) to condense water in ionized air had all been concerned with the negative ion; to catch the positive ions the expansion ratio had to exceed a limit of about 1.31. Wilson then studied the conductivity of air until 1904 and did some research about atmospheric electricity and thunderstorms. It was not until 1910 that he again worked actively at condensation phenomena which led to his discovery of the Wilson Cloud chamber. As the corpuscular nature of α and β radiations was established, he had in view the possibility that the track of an ionizing particle might be made visible and photographed by condensing water on the ions which it liberated.
Much time was spent in making tests of the most suitable form of expansion apparatus and in finding an efficient means of instantaneous illumination of the cloud particles for the purpose of photographing them. In the spring of 1911 tests were still incomplete, but it occurred to me one day to try whether some indication of the tracks might not be made visible with the rough apparatus already constructed. The first test was made with X-rays, with little expectation of success, and in making an expansion of the proper magnitude for condensation on the ions while the air was exposed to the rays I was delighted to see the cloud chamber filled with little wisps and threads of clouds – the tracks of the electrons ejected by the action of the rays. The radium-tipped metal tongue of a spinthariscope was then placed inside the cloud chamber and the very beautiful sight of the clouds condensed along the tracks of the α-particles was seen for the first time.
In April 1911, he published some rough photographs of his find. He improved the apparatus until early 1912 and published a paper in June 1912 showing beautiful and precise photographs of alpha and beta radiations. Further details here.
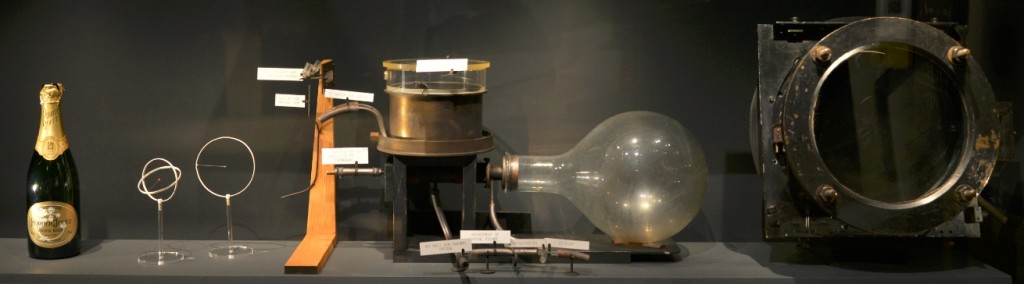
Pictures from the original paper of Wilson :
Two Geiger counter were placed above and below a cloud chamber, so that any cosmic ray passing through the two counters would also pass through the chamber. By a relay mechanism, the electric impulse from the coincident discharge of the counter was made to actuate the expansion of the cloud chamber, which was made so rapid that the ions produced by the ray had no time to diffuse much before the expansion was complete. The chamber was placed in a water-cooled solenoid giving 3,000 gauss. Having made the apparatus ready, one waited for a cosmic ray to arrive and take its own photograph. Instead of a small fraction of photograph showing a cosmic ray track, as when using the method of random expansion, the counter-controlled chamber yielded a cosmic ray track on 80% of the photographs. The first photographs by this new method were made in the early summer of 1932.
1939: Diffusion Cloud Chamber
La chambre à brouillard à diffusion mis au point par Langsdorf en 1939 permet de s’affranchir du fonctionnement « pulsé » des chambres à expansion en créant la couche sursaturée de manière permanente en utilisant de très basses températures : les particules deviennent alors visibles de manière continue dans le temps ce qui est idéal pour étudier la radioactivité émis par des sources. L’inconvénient des chambres à diffusion c’est qu’elles ne peuvent fonctionner que sur un plan horizontal (la gravité stabilisant la couche sursaturée) et donc ne peuvent être utilisées pour étudier le rayonnement cosmique (qui vient très majoritairement perpendiculairement au plan de sensibilité de la chambre). Diffusion cloud chambers were used with accelerators, as the incoming particle are produced in a horizontal plan. But cloud chambers had their limitations for research purposes. They were too small for use at large accelerators. The liquid density wasn’t sufficient to interact with a large number of highly energetic particles . It also had a slow cycle (for the expansion chamber) : the process of reactivating the cloud chamber took too long compared to the accelerator cycles. In 1952, D. Glaser build a bubble chamber which solved these problem.
This chamber had significant advantages of stability and building simpleness over the expansion ones. It had, though, various drawbacks, among which Langsdorf highlighted the following: 1. It required the working area to be horizontal, an inconvenience for the study of cosmic rays, which mostly hit vertically . 2. The large horizontal dimensions of the chamber complicated the application of strong and uniform magnetic fields for track deflection. 3. The limited supply of vapour made the use of strong ionization sources difficult. 4. Because of the continuous supersaturation, any radioactive source placed inside the chamber would constantly
1940-1950: Scintillator, Photomultiplier
1949 : Nuclear Emulsion, Kodak NT4
The method of recording the tracks of charged particles in photographic plates is based upon two achievements, the photographic emulsion and the microscope, which are the result of hundred years of research and industrial experience. Radioactivity was photographed by Becquerel in 1896 using a photographic plate. Nuclear emulsions are similar to optical photographic emulsions. They contain AgBr crystals embedded in gelatin to which small amounts of sensitizing agents have been added. The AgBr content is as much as four times (i.e. 80% AgBr) greater than in optical film. Also the crystals are much smaller (developed grain 0.1 – 0.6 µm) and well separated. The emulsions come in thicknesses from a few µm up to 1 mm. Nuclear radiation passing through the emulsion causes ionization and excitation which activates the AgBr crystals, producing a latent image of the particle path. Upon development the activated crystals serve as centers for further reduction of silver, leading to visible grains.
The emulsions before 1949 did not record the track of a particle if its specific ionization was less than about four time the minimum value (i.e the value characteristic of particles of charge e moving with a velocity closely approaching that of light). As a result, the majority of the fast particles, associated with disintegrations of great energy produced by cosmic radiation, left no visible tracks. Phenomena of great interest and importance were escaping observation, and it became necessary to produce emulsion of greater sensitivity.
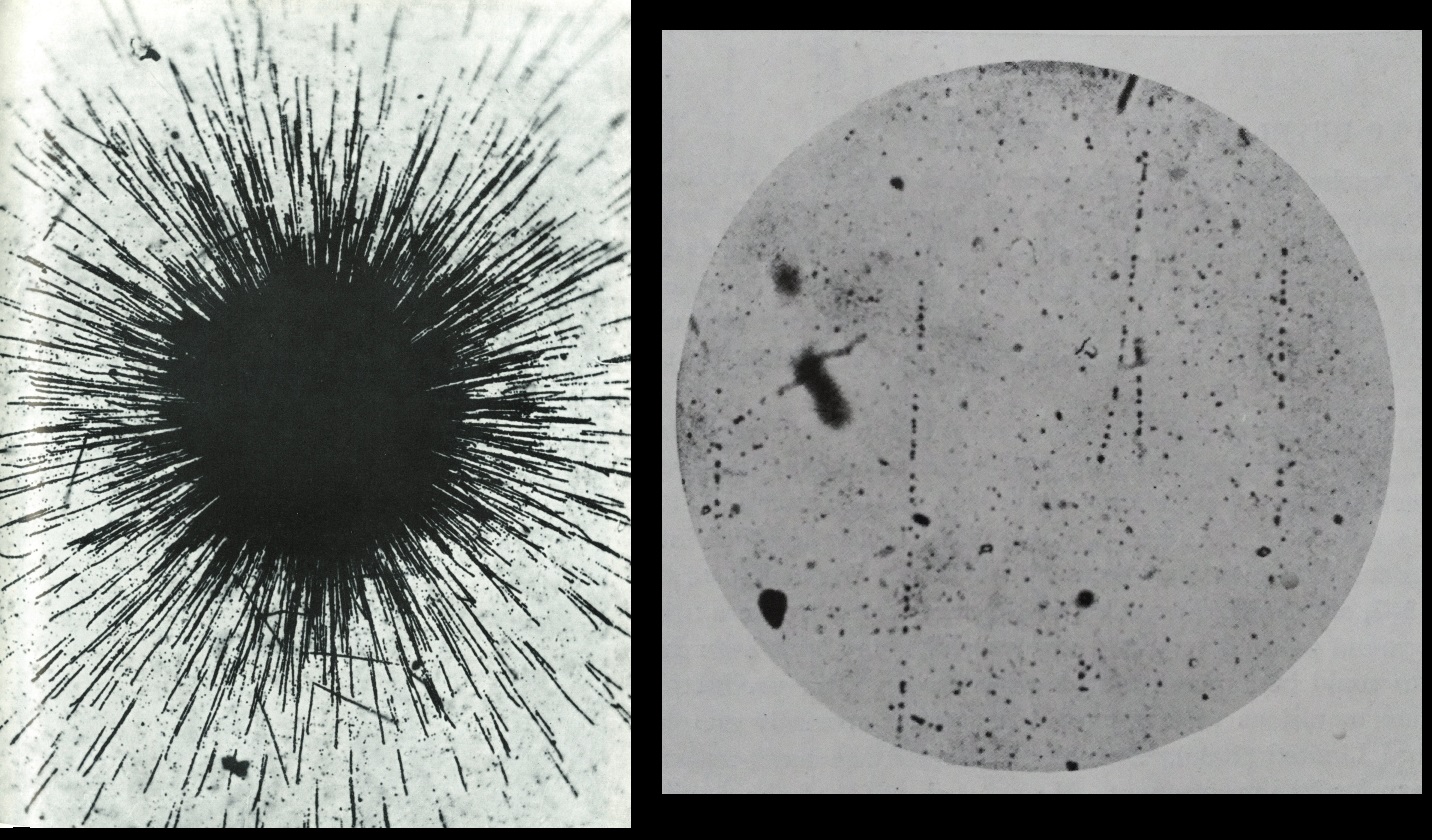
Left : Radium salt on the surface of the photographic plate. The diameter of the core is about 110 μm (1947). Right : tracks of protons in a plate. The length of tracks is about 600 μm (1932).
In 1949, Kodak (and latter Ilford) produced a nuclear emulsion which was sensitive to particles at minimum ionization, by increasing the concentration of halide and lowering the size of grains.
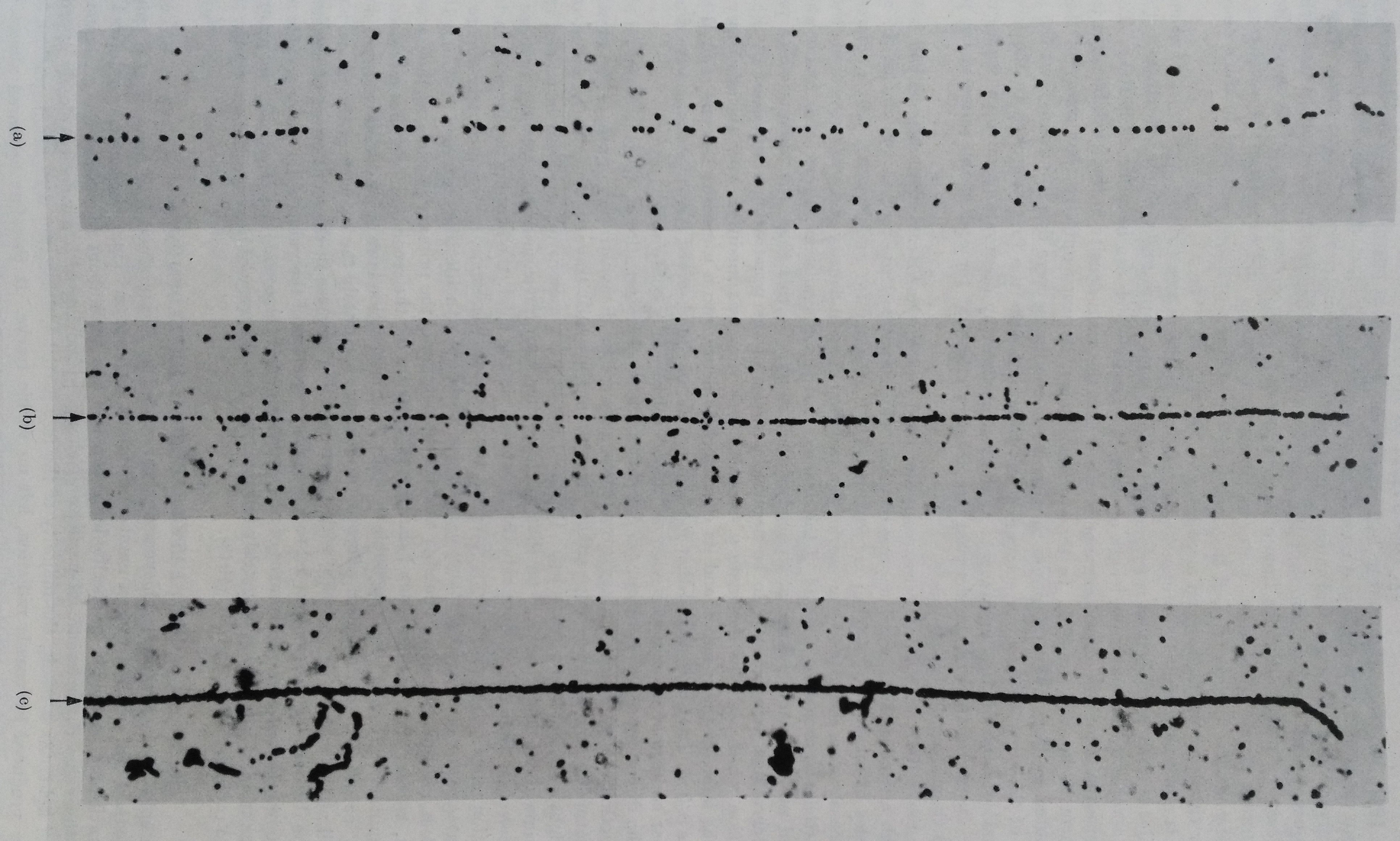
Tracks in different emulsions of protons approaching the end of their range (they have the same energy). (a) Ilford ‘half tone’ emulsion 1939. This emulsion was produced for general photographic work. (b) Ilford C2 emulsion 1946. The greatly increased grain density in the track is clearly visible. Tracks can be distinguished in this emulsion if the specific ionization of the particle is about four time the minimum. (c) Kodak NT4 by Berriman. This was the first ‘electron sensitive’ emulsion and gave recognizable tracks of charged particles of all velocities. The tracks of the proton is almost continuous. The proton has produced several slow δ-rays, in left bottom : the previous emulsions can’t show such low energy events.
With minimum ionization sensible emulsion, the study of particles tracks with photographic plate was possible. By counting the grain density along the tracks, which is proportional to the energy loss dE/dx of the particle, and by measuring the range, the angles of (multiple coulomb) scattering it’s possible to determine the characteristics of the particle (mass, charge, velocity). Example of electrons in an electron sensitive emulsion.
The methods of manufacturing emulsions of such greater sensitivity was a closely guarded secret. There are two distinct ways in which nuclear emulsions can be used. Firstly, they may be placed in the path of particles from an accelerator or in the cosmic radiation and the interactions which these particles produce in the emulsion can be studied in detail. Nuclear emulsion possesses one most significant advantage as it’s capable of extraordinarily high spatial resolution : events separated by a few microns can be resolved. In a diffusion cloud chamber, the natural diffusion of ions don’t permit to have such accuracy with a photograph, the spatial resolution is roughly about 500 μm. Bubble chamber can have a resolution of about 6 μm.
The relatively small size and weight of emulsions enable them to be carried to great heights by means of free balloons or in mountains. For experiments at high altitude, the stacks commonly employed in 1952 were build up of emulsions ~ 600 μm thick, a thickness which corresponds in stopping power ~ 1 m of normal air. The overall dimensions of the stack being 15 x 10 x 2.5 cm. Such a stack is equivalent in stopping power to a Wilson chamber operating with air at a pressure of one atmosphere, of approximate dimensions 300 x 200 x 50 m (this huge proportion come from the density of emulsions which are about 4 g/cm3 , whereas the density of air is 4000 time less. Original article). Other articles about the technique : 1 2.
To summarize about the wonderfulness of emulsions, the heavy density of this medium can slow down a particle a thousand of times more than air and this allow to see the complete life of a particle : from it’s birth at high energy (hundred of MeV) to its end at very low energy (keV) and only in a stack of emulsion few centimeter thick. In a cloud chamber, it would be impossible to see this event ; for example the size of the machine would be about 75 m to see a complete 100 MeV proton track.
In 1947, the pion π was discovered in an emulsion by C.F Powell, who was rewarded a nobel Prize for this discovery and the development of the nuclear emulsions.
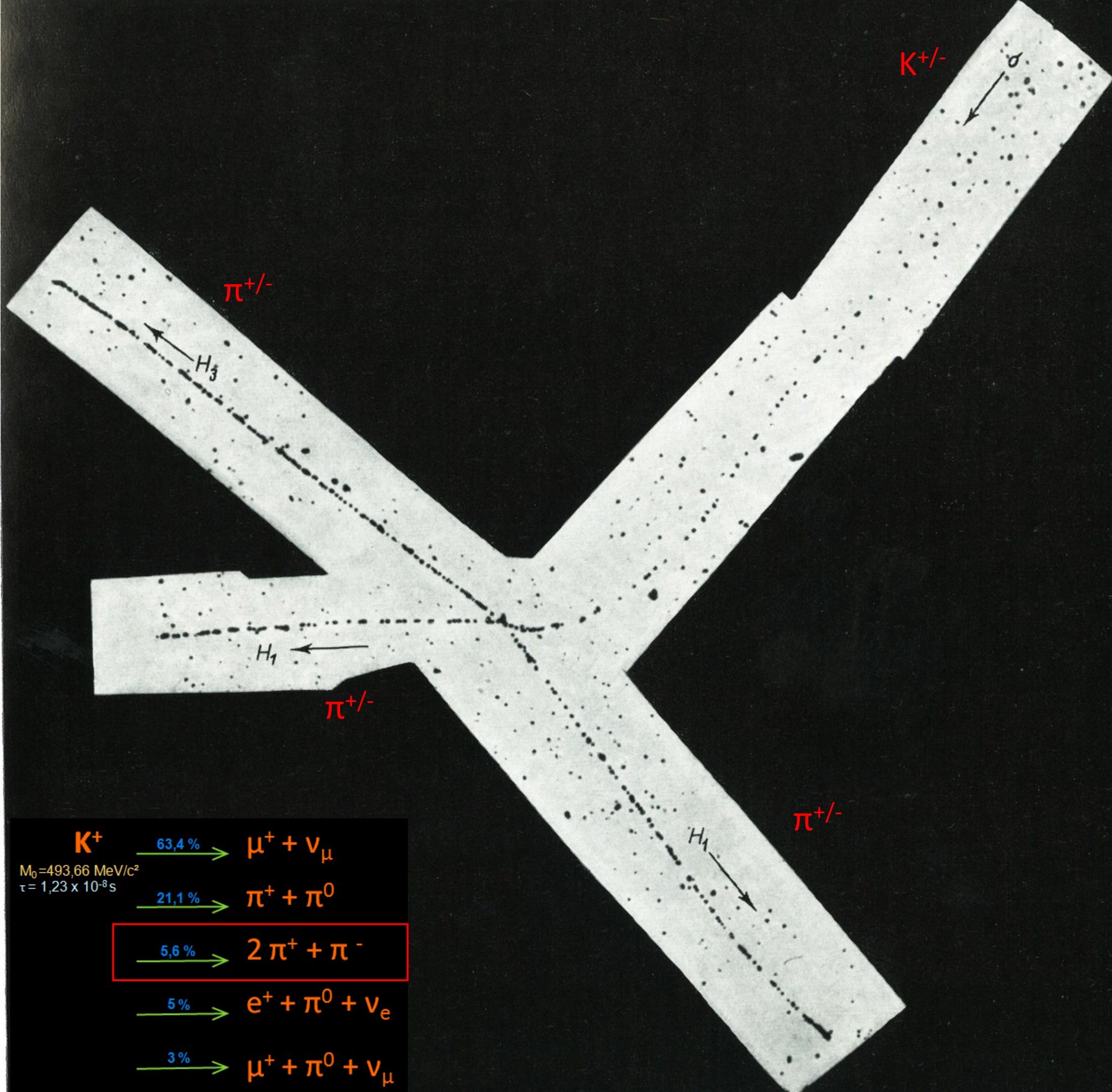
1947 : First photograph of a « new » particle, a kaon, decaying to 3 pions. Nuclear emulsion exposed in mountain at high altitude. The change in grain density as the kaon approaches the end of its range, and the increased scattering are clearly displayed.
The discovery of the π pion in 1947, the elucidation of the characteristics of its decay, and its observed production in high-energy nuclear interactions, gave a clear demonstration of one of the most powerful features of the photographic method. Many π must traverse Wilson chambers, or decay ‘in flight’ without being recognized. On the other hands, π are arrested in a solid emulsion in a time about 2000 times shorter that when moving, with the same initial velocity in a gas at normal pressure. Since they commonly decay ‘at rest’ in the solid, the striking features of their transmutation, such as the constancy in the range of the secondary μ muon, are immediately apparent. The discovery of the pion led to a general recognition of the value of the photographic method, and to its widespread use for studies of nuclear physics and the cosmic radiation. Among many observations of great interest which quickly followed was the discovery of heavy nuclei among the primary cosmic radiation (1948). C.F Powell The Study of Elementary Particles by the Photographic Method 1959.
1952 : Bubble Chamber
Bubble chambers used an overheated liquid instead of a supersaturated gas for track formation, therefore giving more stability and allowing easy resets and triggering that reduced the background signal
Unlike the Cloud Chamber, the Bubble Chamber could not be triggered, i.e. the bubble chamber had to be already in the superheated state when the particle was entering. It was therefore not useful for Cosmic Ray Physics, but as in the 50ies particle physics moved to accelerators it was possible to synchronize the chamber compression with the arrival of the beam.
1962 : Spark Chamber
For the a chronology of particles physics, you should read this document.